Green aviation
COURTESY : physicsworld.com/
Commercial air travel has changed a lot since the first aeroplane took passengers around a century ago. Brian Tillotson explores the future challenges to make aviation greener
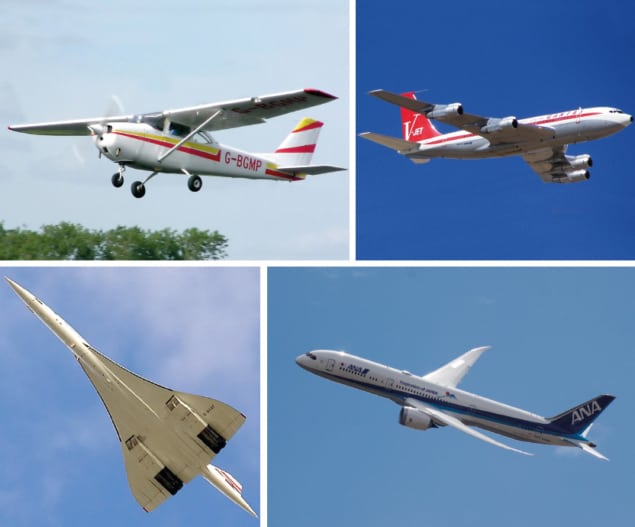
Once upon a time, air travel was risky, costly and highly polluting. But these days modern airliners are the safest way to travel long distances, as well as being cheaper, quieter and more fuel-efficient than their predecessors. If you need to cross the US, a seat on an Airbus will get you there on less than half the fuel of an average American car – and save you three days.
Unfortunately, this efficiency is a double-edged sword, encouraging more and more people to fly or send cargo by plane. Aviation’s contribution to the world’s CO2 emissions has risen from zero to 2% within the last century despite the fact that the litres of fuel burned per passenger-kilometre has plummeted – from 10.3 l/100 passenger-km for the De Havilland Comet 4 in 1958, when commercial flight with jet engine aircraft was new, to 2.42 l/100 passenger-km with today’s Airbus A330neo-900. Furthermore, with around 107,000 commercial flights taking place every day prior to the COVID-19 pandemic, noise pollution from airports is a huge concern.
Aviation’s contribution to the world’s CO2 emissions has risen from zero to 2% within the last century despite the fact that the fuel burned per passenger-kilometre has plummeted
Green solutions
So, what can be done to make aviation greener? Well let’s consider Boeing’s 787 Dreamliner – a modern airliner that looks much like its noisier, less-efficient ancestor, the 707. Each has a cylindrical fuselage with a square-root-of-x nose profile, wings swept back about 35°, and jet engines sticking forward from under the wings. This shape is set by the laws of mechanics and by compressible fluid flow.
Look closer, however, and differences emerge. The Dreamliner’s engines are fatter in front, so they have a high “bypass ratio” – they put less kinetic energy into the airstream for the same amount of thrust. The engine exhaust nozzles are scalloped with a chevron shape, which creates vortices in the exhaust fumes. When high-speed exhaust mixes with the slower-moving air, the vortices make less noise than the chaotic mixing of earlier engines.
More subtly, the leading edge of the 707’s aluminium wing is straight but the Dreamliner’s is curved, which reduces fuel-wasting drag. Although the shape is too complex to be affordably built with aluminium, it is made possible thanks to a composite material – carbon-fibre-reinforced plastic (CFRP). Besides allowing aircraft components such as the wings, tail and fuselage to have a wide variety of shapes, CFRP is stiffer and has a higher strength-to-weight ratio than aluminium or steel. These factors make the aeroplane lighter, so less fuel is needed to get it off the ground and keep it airborne.
One challenge of CFRP is in the factory, where it starts as a flexible fabric or tape infused with uncured epoxy resin. This is laid on moulds by hand or by automated tape-laying machines. To make it rigid, it then has to be “cured” or baked in an autoclave at temperatures of up to 180 °C, depending on the blend of CFRP. The problem is that ensuring that every region of the part stays at exactly the right temperature is tricky, calling for careful process control and sometimes for instruments embedded within the fabric. To verify that every ply of material (there can be as many as 100 layers) lies smoothly on the one below it with no wrinkles, voids or delaminations, aircraft manufacturers use inspection methods, such as machine-vision, for automatic checks while the tape is being applied, and X-ray and ultrasound to verify each part before it’s built into a larger structure.
When lightning strikes
A big issue for CFRP is that it has a very high resistance, which is a problem when a typical commercial airliner is struck by lightning at least once a year. A bolt can carry as much as 200 kA of electrical current, which enters at one location, such as a wingtip, and exits at another, like the tail. Aeroplanes that have skin and sub-structure of mostly aluminium easily handle the current and the heat it produces at the point of attachment: engineers only need to include good conductive paths between components, skins thick enough to prevent melt-through, and internal sealant over gaps or joints to prevent arcs and sparks.
Part of the problem is that CFRP conducts electricity anisotropically. Current flows fairly easily along the fibres, which are 2000 times less conductive than copper but 30 times more conductive than silicon. However, current flows poorly between the fibres or between ply layers. Electrical potential from a lightning strike may therefore arc through the air to get around non-conductive zones, or the current may heat fibres and create a spark of vaporized material – either of which could cause a fire in a fuel tank.
Governments and manufacturers have therefore developed ways to avoid arcs and sparks. Metal foils, for example, are used on the outside of a plane to guide lightning current and protect composite skins. Other techniques include using dielectric material to isolate current and direct it away from sensitive components, and minimizing resistance in fastener installations. There are also laboratory experiments and software that can simulate lightning current, allowing us to get a better theoretical and experimental understanding of lightning attachment, arc conditions and ignition processes. Engineers can then use this knowledge to design aircraft with large margins of safety above the strongest lightning strike. These efforts have paid off. Aircraft built with CFRP wings and fuselages are routinely struck by lightning, just like their aluminium siblings, but none has had an ignition. The challenge now is to reduce the weight of these solutions so fuel economy gets even better.
While heating and arcing are direct effects of a lightning strike, it can also cause indirect effects by inducing voltage, current or force in components where the lightning current doesn’t reach. For example, a fast-changing lightning current can induce a voltage that flips a bit in nearby electronics, or it can induce eddy-current forces so strong they bend or break some components. Designers use modelling and testing to verify that indirect effects cause no problems, or that counter-measures such as shunt diodes or shielded cables keep a current spike from reaching a sensitive component.
Sweeping wings
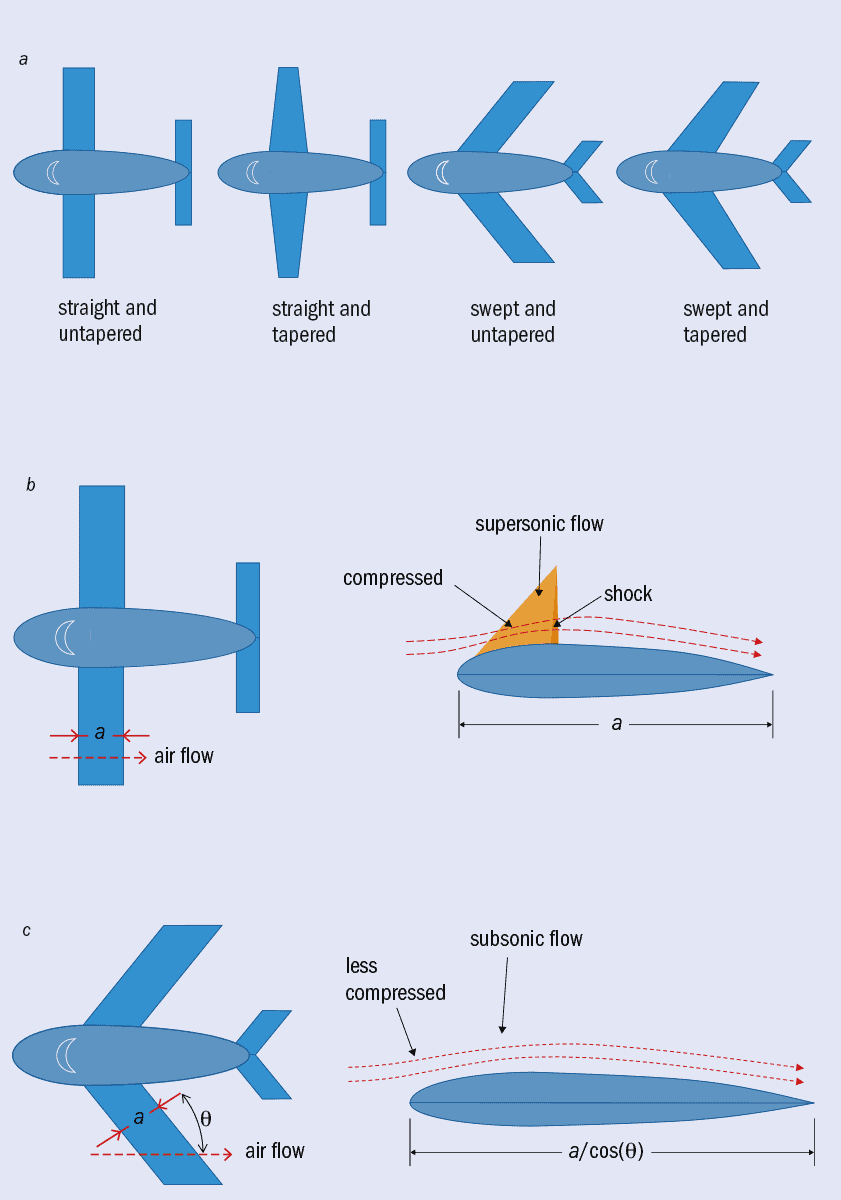
On an aeroplane, the wings’ job is to lift the craft against gravity. It does this by deflecting air downward, which imparts downward momentum to the air and an equal upward momentum to the wings. The structure inside the wing transfers the force to the fuselage (the body of the plane) thereby allowing the aircraft to fly.
(a) Early planes featured straight, thick wings – indeed, some modern small aircraft still do. While these are structurally efficient, requiring little mass to safely transfer lifting force to the body, a tapered wing is even better. The structure is lighter than for straight wings because most lift is produced by the thick region near the fuselage, meaning it only has to transfer that lift a short distance.
A plane can also be made more stable if there is slight sweep back on the leading edge. For a plane flying straight, the wings have equal cross-section to the airstream. When the craft twists to the right, perhaps from a gust of turbulence, a swept left-wing presents a larger cross-section to the wind than the right wing. This helps the aeroplane quickly straighten out after small disturbances.
For planes with jet engines, the wings are swept back even further than for their slower, non-jet-engine counterparts – in some cases they can even be angled at more than 40°.
Jet engines allow planes to fly near the speed of sound, at transonic speeds. Air flowing over curved or thick parts of the plane must move even faster than the speed the plane is travelling, often meaning it reaches supersonic speeds (faster than the speed of sound). So when that supersonic airflow moving over those thicker parts of the wing collides with slower moving air, it creates shockwaves. A shock irreversibly transforms the air’s kinetic energy into heat and that loss of energy creates lots of drag.
The engineers who built early jets solved the problem by sweeping the wings. (b) With a straight wing’s profile, in the thick region, airflow is supersonic. (c) With a swept wing, the air follows a gentler path. Its arrival at the thick part of the wing is delayed by a factor of 1/cos(θ), where θ is the sweep angle. Air farther from the wing has more time to shift out of the way, so air near the wing has a wider “channel”. It needn’t go supersonic to get around the wing.
The next generation
Though the shape of airliners has changed little over the last 60 years, the cantilevered swept-wing shape may, over the next decade or two, finally give way to a new design thanks to NASA and companies like Boeing, which are developing the “transonic truss-braced wing” that will exhibit little or no sweep.
Conventional truss-braced wings with no sweeping V-shape are an old idea and the design is still popular for small aeroplanes. In fact, the world’s best-selling aircraft, the four-seat Cessna 172, has a straight truss-braced wing. Until now, however, no jet airliner has used an unswept wing, truss-braced or not, because it produces too much drag at transonic speeds, those that are near or at the speed of sound (see box above). Airliners usually travel at Mach 0.8–0.85, where the Mach number is the fraction of the speed of sound in the local atmospheric condition (343 m/s in dry air at 20 °C) – typically the actual speed is 246–271 m/s (885–974 km/h) depending on air temperature.
New, thin airfoils made possible by CFRP, plus structural bracing by thin CFRP trusses, finally allow for wings with little or no sweep that can travel at these speeds without producing too much drag. The advantages of an unswept wing include the fact that it is structurally more efficient than a swept-back wing. To have a given wingspan, a swept wing must be longer than an unswept one. The wing’s lift then acts with a longer moment arm, which increases the bending moment on the wing’s structure. To resist this, the structure of the swept wing must be stronger, and therefore heavier, than an unswept one.
The external truss also reduces stress on the wing’s internal structure so the wing can be thinner top-to-bottom, giving it a small frontal cross-section and thereby reducing drag. To cut drag more, the wing is narrower front-to-back than a traditional wing, which lets laminar flow cover a larger fraction of the wing surface. The wing is also longer relative to its front-to-back width, or “chord”, and this higher aspect ratio reduces the energy lost when high-pressure air from below the wing flows around the wingtip to the low-pressure region above the wing. An upward-folding tip allows this long-winged aircraft to fit into the small gates of regional airports.
Electric aeroplanes
Another way to make planes greener is to develop hybrid propulsion, rather like we now have hybrid cars. The idea is that jet turbines would boost power for take-off while batteries or fuel cells would provide steady power for cruise. Of course, it would be even better if aeroplanes could fly purely on renewable energy (such as solar or hydro) or nuclear power, which emit no carbon.
Alas, an all-electric airliner to replace today’s planes is not on the cards. Batteries have a specific energy (measured in joules per kilogram) that is orders of magnitude lower than the specific energy of hydrocarbon fuel. This figure will improve, but not enough. That’s because when weight matters, as it does in aeroplanes, batteries will always suffer from the fact that they have to carry their own oxidizer, whereas fuel-burning engines use oxygen from air. Therefore, a battery-powered aeroplane or helicopter can only work over tens or perhaps hundreds of miles, not over the thousands of miles where an aeroplane’s speed makes it most useful.
A battery-powered aeroplane or helicopter can only work over tens or perhaps hundreds of miles, not over the thousands of miles where an aeroplane’s speed makes it most useful
There are a few prospects on the horizon for getting around the fundamental limits of batteries. One option is to use lasers or microwaves to transmit energy from the ground to aircraft in flight. The beam of photons creates voltage and current in dipole antenna elements or photovoltaic arrays on the aircraft, with diodes siphoning off the power to run motors or electronics. This has been done at a small scale. One of the best demonstrations was by the SHARP project in Canada back in 1987 using microwaves, but more recent efforts include Lockheed Martin and LaserMotive (now PowerLight) flying an unmanned aerial vehicle (UAV) using laser beams. However, the method faces regulatory and cost challenges before it can scale up to commercial flight.
As for solar-powered aircraft, they do already exist, but because the sunlight is so weak, these fragile aircraft fly slowly and with little payload. A third option is to recharge aircraft in flight, just as aerial tankers transfer fuel to military aircraft today. It’s plausible for long-range all-electric flight, but technical challenges remain.
From super to hypersonic airliners
Another development that we could see over the next two or three decades is hypersonic travel, which means craft flying at Mach 5 (6126 km/h) or faster. This kind of speed could get you from Los Angeles to Beijing in no more than two hours. Supersonic airliners – the Concorde and the Tupolev 144 – only flew at half that speed and gave off huge amounts of CO2. They were also horribly noisy at take-off and landing, and emitted sonic booms during cruise. Both are now out of operation.
Hypersonic airliners will do better. These new aircraft will likely use hydrogen – produced by electrolysis using non-fossil energy sources – or low-carbon fuel like methane. With such light molecules, the fuel and air can rapidly mix, which is vital because air moves through the engine so fast it spends only a few milliseconds in the combustion zone.

Sonic boom is less of a problem now, too. NASA is already developing low-boom aircraft designs, which have a long, sharp nose that reduces the area over which air is compressed to form the leading shockwave. They also keep protuberances, like the engine intake, on top of the fuselage so shockwaves produced there go up, not downward to bother people.
What’s more, thanks to a quirk of the atmosphere, most acoustic energy from hypersonic planes will never reach Earth. If you imagine a sonic boom heading out diagonally down from an aircraft, the sound will meet steadily warmer air as it moves. Given that sound and shockwaves travel faster in warm air, the sonic boom will refract – its path will curve and become more horizontal as it moves lower. So apart from shockwaves that are heading almost straight down, the path eventually curves upward, avoiding the ground altogether. And because hypersonic aircraft fly at 27,000 to 30,000 m – more than twice as high as today’s airliners, because higher altitudes mean thinner air and therefore less drag – nearly 100% of the sonic boom refracts away from the Earth.
Despite these benefits, several challenges of hypersonic flight must be overcome. One is the fact that air at the nose and leading edge of a hypersonic plane can get hotter than 1300 K because the air’s kinetic energy becomes heat energy when it runs into the perpendicular surface and stops. At those temperatures, CFRP, which has revolutionized today’s airliners, simply doesn’t hold its strength. Fortunately, titanium alloys and other advanced, lightweight materials – some with honeycomb structures to reduce heat transport – are being developed to solve this problem.
Another issue is ionizing radiation. When a galactic cosmic-ray particle strikes a nucleus in the upper atmosphere, the nucleus shatters into a spray of secondary particles. The trails of these secondaries create conductive paths in semiconductor electronics. Today’s planes fly at altitudes of 9000–13,000 m, where the secondaries are spread out, so any one electronic device sees only a small effect. But for a hypersonic plane flying at 30,000 m, the secondaries will be tightly clumped still, creating a much stronger radiation environment. It wouldn’t be a problem for passengers; a flight will be so short that the total dose would be lower than in today’s flights. But electronics will have trouble. A single chip may be hit by many secondaries at the same time, and such a sudden rise in conductivity can cause errors in a semiconductor chip, or even damage it permanently. Costly solutions do exist for spacecraft, such as physical shielding or electronics made of expensive materials with large band gaps. The challenge is to devise solutions for aircraft that are affordable and lightweight.
Urban aviation
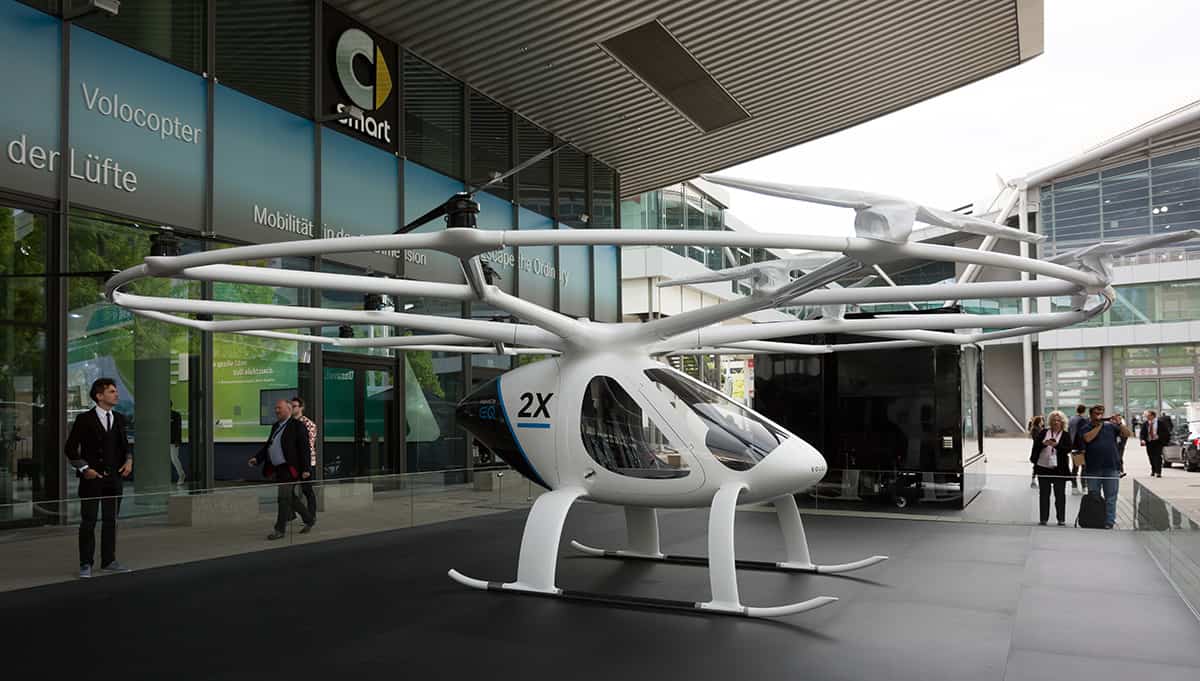
With towns and cities suffering from rising congestion on the roads, there is a growing demand for an aircraft that can land vertically in a small space, load one or two people or a van-sized cargo, and fly above the clogged highways to another spot in the same city. That’s where battery-powered helicopters could be the answer. With four or more rotors, these electric “rotorcraft” will soon be carrying cargo, and perhaps passengers, in cities. Their short range – a few dozen miles – is a perfect match for urban flight.
This new family of aircraft has many environmental benefits. Because they fly at about 320 km/h and use uncongested routes, each aircraft can make as many trips per day as, say, 10 cars or trucks. If that happens, it gets 10 vehicles off the roads, making our commute a little easier. Each aircraft would also mean that 10 cars or trucks wouldn’t need to be built, saving energy and materials. Of course, being electric-powered, the aircraft emit no CO2 in cities where electricity comes from non-fossil sources. It also helps keep engine noise tolerable.
Challenges remain, however. For a two-person taxi service, the aircraft would have to be recharged quickly. The 15-minute charging time of today’s batteries would need to be shortened, or ways would have to be found to quickly swap depleted batteries for charged ones. Batteries would go through up to 20 deep discharge cycles per day, so they would reach end-of-life in a few months as the electrochemical process involved in a rechargeable battery isn’t totally reversible. That’s because with each charge–discharge cycle, some atoms from the electrodes or the electrolyte go to the wrong place, eventually weakening the battery and making it potentially develop too much leakage current to hold charge for long. Discarding or refurbishing the batteries at the required rate will therefore be a challenge.
Helicopters are also noisy, as is any vehicle with many small rotors. There are ways to make them quieter, like ducts around the rotors, but these have costs and trade-offs.
Finally, the density of air traffic would be unprecedented; a thousand aircraft simultaneously flying in an area the size of Los Angeles or London will require a new, and probably automated, approach to air traffic control. Computers, not humans, would have to pilot these vehicles and co-ordinate with traffic control.
In essence, the commercial aviation sector is continuing to refine and develop traditional aircraft as it has throughout its 100-year-old history, constantly seeking to reduce costs and lower their impact on the environment. But there are now new directions for the industry too, in the form of super-efficient new airliners that will look quite different, electric-powered rotorcraft that revolutionize urban transport (see box above), and hypersonic aeroplanes that protect the world’s environment while shrinking the distance between its people. Within decades, air travel may look very different to now.